Gravitational Waves Discovered at Long Last
Ripples in space-time have been detected a century after Einstein predicted them, launching a new era in astronomy.
Ripples in space-time caused by the violent mergers of black holes have been detected, 100 years after these “gravitational waves” were predicted by Albert Einstein’s theory of general relativity and half a century after physicists set out to look for them.
The landmark discovery was reported today by the Advanced Laser Interferometer Gravitational-Wave Observatory (Advanced LIGO) team, confirming months of rumors that have surrounded the group’s analysis of its first round of data. Astrophysicists say the detection of gravitational waves opens up a new window on the universe, revealing faraway events that can’t be seen by optical telescopes, but whose faint tremors can be felt, even heard, across the cosmos.
“We have detected gravitational waves. We did it!” announced David Reitze, executive director of the 1,000-member team, at a National Science Foundation press conference today in Washington, D.C.
Gravitational waves are perhaps the most elusive prediction of Einstein’s theory, one that he and his contemporaries debated for decades. According to his theory, space and time form a stretchy fabric that bends under heavy objects, and to feel gravity is to fall along the fabric’s curves. But can the “space-time” fabric ripple like the skin of a drum? Einstein flip-flopped, confused as to what his equations implied. But even steadfast believers assumed that, in any case, gravitational waves would be too weak to observe. They cascade outward from certain cataclysmic events, alternately stretching and squeezing space-time as they go. But by the time the waves reach Earth from these remote sources, they typically stretch and squeeze each mile of space by a minuscule fraction of the width of an atomic nucleus.
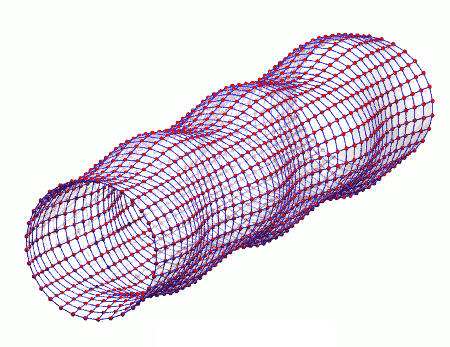
M. Pössel/Einstein Online
Gravitational waves alternately stretch and squeeze space-time both vertically and horizontally as they propagate.
Perceiving the waves took patience and a delicate touch. Advanced LIGO bounced laser beams back and forth along the four-kilometer arms of two L-shaped detectors — one in Hanford, Wash., the other in Livingston, La. — looking for coincident expansions and contractions of their arms caused by gravitational waves as they passed. Using state-of-the-art stabilizers, vacuums and thousands of sensors, the scientists measured changes in the arms’ lengths as tiny as one thousandth the width of a proton. This sensitivity would have been unimaginable a century ago, and struck many as implausible in 1968, when Rainer Weiss of the Massachusetts Institute of Technology conceived the experiment that became LIGO.
“The great wonder is they did finally pull it off; they managed to detect these little boogers!” said Daniel Kennefick, a theoretical physicist at the University of Arkansas and author of the 2007 book Traveling at the Speed of Thought: Einstein and the Quest for Gravitational Waves.
The detection ushers in a new era of gravitational-wave astronomy that is expected to deliver a better understanding of the formation, population and galactic role of black holes — super-dense balls of mass that curve space-time so steeply thateven light cannot escape. When black holes spiral toward each other and merge, they emit a “chirp”: space-time ripples that grow higher in pitch and amplitude before abruptly ending. The chirps that LIGO can detect happen to fall in the audible range, although they are far too quiet to be heard by the unaided ear. You can re-create the sound by running your finger along a piano’s keys. “Start from the lowest note on the piano and go to middle C,” Weiss said. “That’s what we hear.”
NSF
Audio: The “chirp” of gravitational waves recorded by the LIGO team.
Physicists are already surprised by the number and strength of the signals detected so far, which imply that there are more black holes out there than expected. “We got lucky, but I was always expecting us to be somewhat lucky,” saidKip Thorne, a theoretical physicist at the California Institute of Technology who founded LIGO with Weiss and Ronald Drever, who is also at Caltech. “This usually happens when a whole new window’s been opened up on the universe.”
C. Henze/ NASA
Video: A simulation of two black holes merging and the resulting emission of gravitational radiation.
Eavesdropping on gravitational waves could reshape our view of the cosmos in other ways, perhaps uncovering unimagined cosmic happenings.
“I liken this to the first time we pointed a telescope at the sky,” saidJanna Levin, a theoretical astrophysicist at Barnard College of Columbia University. “People realized there was something to see out there, but didn’t foresee the huge, incredible range of possibilities that exist in the universe.” Similarly, Levin said, gravitational-wave detections might possibly reveal that “the universe is full of dark stuff that we simply can’t detect in a telescope.”
The story of the first gravitational-wave detection began on a Monday morning in September, and it started with a bang: a signal so loud and clear that Weiss thought, “This is crap. It’s gotta be no good.”
Fever Pitch
That first gravitational wave swept across Advanced LIGO’s detectors — first at Livingston, then at Hanford seven milliseconds later — during a mock run in the early hours of Sept. 14, two days before data collection was officially scheduled to begin.
The detectors were just firing up again after a five-year, $200-million upgrade, which equipped them with new noise-damping mirror suspensions and an active feedback system for canceling out extraneous vibrations in real time. The upgrades gave Advanced LIGO a major sensitivity boost over its predecessor, “initial LIGO,” which from 2002 to 2010 had detected “a good clean zero,” as Weiss put it.
When the big signal arrived in September, scientists in Europe, where it was morning, frantically emailed their American colleagues. As the rest of the team awoke, the news quickly spread. According to Weiss, practically everyone was skeptical — especially when they saw the signal. It was such a textbook chirp that many suspected the data had been hacked.
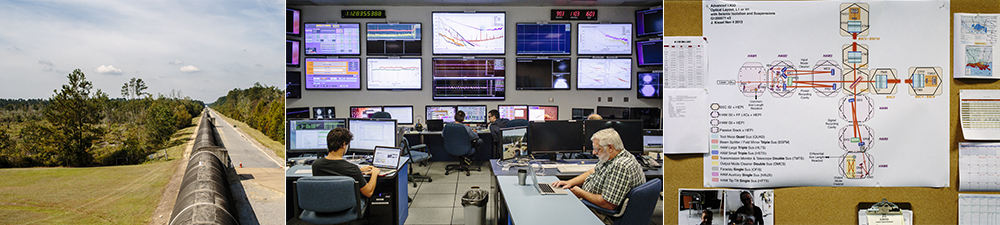
William Widmer for Quanta Magazine
From left: A four-kilometer arm of the LIGO Livingston Observatory, the control room, and a schematic diagram of the detector’s “optical layout.”
Mistaken claims in the search for gravitational waves have a long history, starting in the late 1960s when Joseph Weber of the University of Maryland thought he observed aluminum bars resonating in response to the waves. Most recently, in 2014, an experiment called BICEP2 reported the detection of primordial gravitational waves — space-time ripples from the Big Bang that would now be stretched and permanently frozen into the geometry of the universe. The BICEP2 team went public with great fanfare before their results were peer-reviewed, and then got burned when their signal turned out to have come from space dust.
When Lawrence Krauss, a cosmologist at Arizona State University, got wind of the Advanced LIGO detection, “the first thought is that it was a blind injection,” he said. During initial LIGO, simulated signals had been secretly inserted into the data streams to test the response, unbeknownst to most of the team. When Krauss heard from an inside source that it wasn’t a blind injection this time, he could hardly contain his excitement.
On Sept. 25, he tweeted to his 200,000 followers: “Rumor of a gravitational wave detection at LIGO detector. Amazing if true. Will post details if it survives.” Then, onJan. 11: “My earlier rumor about LIGO has been confirmed by independent sources. Stay tuned! Gravitational waves may have been discovered!”
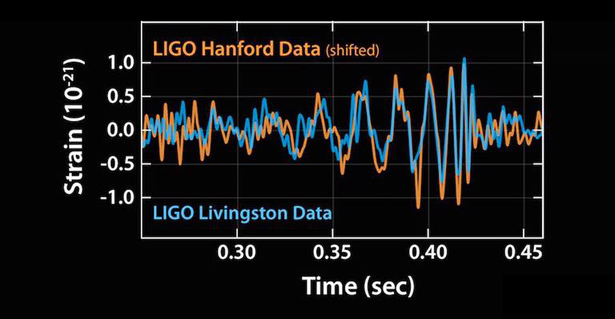
LIGO
The first gravitational wave signal was observed seven milliseconds apart on Sept. 14 at Advanced LIGO’s Hanford and Livingston detectors.
The team’s official stance was to keep quiet about their signal until they were dead sure. Thorne, bound by a vow of secrecy, didn’t even tell his wife. “I celebrated in private,” he said. The team’s first step was to go back and analyze in excruciating detail how the signal had propagated through the detectors’ thousands of different measurement channels, and to see whether anything strange had happened at the moment the signal was seen. They found nothing unusual. They also ruled out hackers, who would have had to know more than anyone about the experiment’s thousands of data streams. “Even the team that does the blind injections have not perfected their injections well enough not to leave behind lots of fingerprints,” Thorne said. “And there were no fingerprints.”
Another, weaker chirp showed up in the weeks that followed.
The scientists analyzed these first two signals as even more swept in, and they submitted their paper to Physical Review Letters in January; it appeared online today. Their estimate of the statistical significance of the first, biggest signal is above “5-sigma,” meaning the scientists are 99.9999 percent sure it’s real.
Listening for Gravity
Einstein’s equations of general relativity are so complex that it took 40 years for most physicists to agree that gravitational waves exist and are detectable — even in theory.
Einstein first thought that objects cannot shed energy in the form of gravitational radiation, then changed his mind. He showed in a seminal 1918 paper which ones could: Dumbbell-like systems that rotate about two axes at once, such as binary stars and supernovas popping like firecrackers, can make waves in space-time.
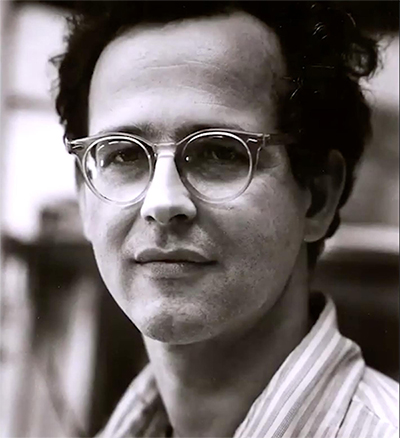
Courtesy of Kip Thorne
Rainer Weiss, a professor of physics at the Massachusetts Institute of Technology, around 1970.
Still, Einstein and his colleagues continued to waffle. Some physicists argued that even if the waves exist, the world will oscillate with them and they cannot be felt. It wasn’t until 1957 that Richard Feynman put that question to rest, with a thought experiment demonstrating that, if gravitational waves exist, they are theoretically detectable. But nobody knew how common those dumbbell-like sources might be in our cosmic neighborhood, or how strong or weak the resulting waves would be. “There was that ultimate question of: Will we ever really detect them?” Kennefick said.
In 1968, “Rai” Weiss was a young professor at MIT who had been roped into teaching a class on general relativity — a theory that he, as an experimentalist, knew little about — when news broke that Joseph Weber had detected gravitational waves. Weber had set up a trio of desk-size aluminum bars in two different U.S. states, and he reported that gravitational waves had set them all ringing.
Weiss’ students asked him to explain gravitational waves and weigh in about the news. Looking into it, he was intimidated by the complex mathematics. “I couldn’t figure out what the hell [Weber] was doing — how the bar interacted with the gravitational wave.” He sat for a long time, asking himself, “What’s the most primitive thing I can think of that will detect gravitational waves?” An idea came to him that he calls the “conceptual basis of LIGO.”
Imagine three objects sitting in space-time — say, mirrors at the corners of a triangle. “Send light from one to the other,” Weiss said. “Look at the time it takes to go from one mass to another, and see if the time has changed.” It turns out, he said, “you can do that quickly. I gave it to [my students] as a problem. Virtually the whole class was able to do that calculation.”
In the next few years, as other researchers tried and failed to replicate the results of Weber’s resonance-bar experiments (what he observed remains unclear, but it wasn’t gravitational waves), Weiss began plotting a much more precise and ambitious experiment: a gravitational-wave interferometer. Laser light would bounce between three mirrors in an L-shaped arrangement, forming two beams. The spacing of the peaks and troughs of the light waves would precisely measure the lengths of the two arms, creating what could be thought of as x and y axes for space-time. When the grid was still, the two light waves would bounce back to the corner and cancel each other out, producing a null signal in a detector. But if a gravitational wave swept across Earth, it would stretch the length of one arm and compress the length of the other (and vice versa in an alternating pattern). The off-alignment of the two light beams would create a signal in the detector, revealing a fleeting tremor in space and time.
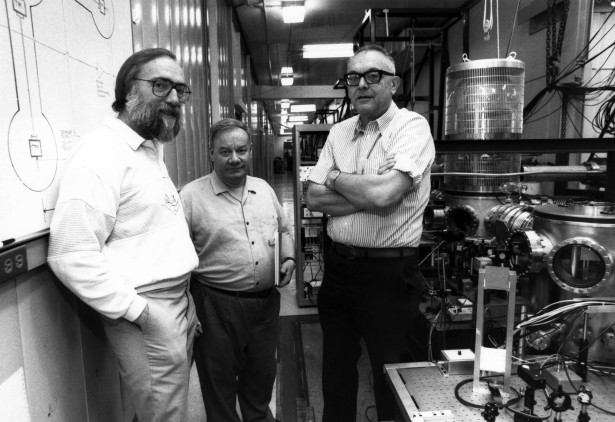
Courtesy of the Archives, California Institute of Technology
From left: Kip Thorne, Ron Drever and Robbie Vogt, the first director of the LIGO project, with a 40-meter prototype of the LIGO detectors at the California Institute of Technology in 1990.
Fellow physicists were skeptical at first, but the experiment soon found a champion in Thorne, whose theory group at Caltech studied black holes and other potential gravitational-wave sources and the signals they would produce. Thorne had been inspired by Weber’s experiment and similar efforts by Russian physicists; after speaking with Weiss at a conference in 1975, “I began to believe that gravitational-wave detection would succeed,” Thorne said, “and I wanted Caltech to be involved.” He had Caltech hire the Scottish experimentalist Ronald Drever, who had also been clamoring to build a gravitational-wave interferometer. Thorne, Drever and Weiss eventually began working as a team, each taking on a share of the countless problems that had to be solved to develop a feasible experiment. The trio founded LIGO in 1984, and, after building prototypes and collaborating with a growing team, banked more than $100 million in NSF funding in the early 1990s. Blueprints were drawn up for a pair of giant L-shaped detectors. A decade later, the detectors went online.
In Hanford and Livingston, vacuums run down the center of each detector’s four-kilometer arms, keeping the laser, the beam path and the mirrors as isolated as possible from the planet’s constant trembling. Not taking any chances, LIGO scientists monitor their detectors with thousands of instruments during each data run, measuring everything they can: seismic activity, atmospheric pressure, lightning, the arrival of cosmic rays, vibrations of the equipment, sounds near the laser beam, and so on. They then cleanse their data of these various sources of background noise. Perhaps most importantly, having two detectors allows them to cross-check their data, looking for coincident signals.
Related Articles:
Searching the Sky for the Wobbles of GravityAn October 2015 interview with LIGO spokesperson Gabriela González on the search for gravitational waves.The Quantum Fabric of Space-TimeImagine the fabric of space-time peeled back layer by layer.
Inside the vacuum, even with isolated and stabilized lasers and mirrors, “strange signals happen all the time,” said Marco Cavaglià, assistant spokesperson for the LIGO collaboration. The scientists must trace these “koi fish,” “ghosts,” “fringy sea monsters” and other rogue vibrational patterns back to their sources so the culprits can be removed. One tough case occurred during the testing phase, said Jessica McIver, a postdoctoral researcher and one of the team’s foremost glitch detectives. It was a string of periodic, single-frequency artifacts that appeared every so often in the data. When she and her colleagues converted the mirror vibrations into an audio file, “you could clearly hear the ring-ring-ring of a telephone,” McIver said. “It turned out to be telemarketers calling the phone inside the laser enclosure.”
The sensitivity of Advanced LIGO’s detectors will continue to improve over the next couple of years, and a third interferometer called Advanced Virgo will come online in Italy. One question the data might help answer is how black holes form. Are they products of implosions of the earliest, massive stars, or do they originate from collisions inside tight clusters of stars? “Those are just two ideas; I bet there will be several more before the dust settles,” Weiss said. As LIGO tallies new statistics in future runs, scientists will be listening for whispers of these black-hole origin stories.
Judging by its shape and size, that first, loudest chirp originated about 1.3 billion light-years away from the location where two black holes, each of roughly 30 solar masses, finally merged after slow-dancing under mutual gravitational attraction for eons. The black holes spiraled toward each other faster and faster as the end drew near, like water in a drain, shedding three suns’ worth of energy to gravitational waves in roughly the blink of an eye. The merger is the most energetic event ever detected.
“It’s as though we had never seen the ocean in a storm,” Thorne said. He has been waiting for a storm in space-time ever since the 1960s. The feeling he experienced when the waves finally rolled in wasn’t excitement, he said, but something else: profound satisfaction.